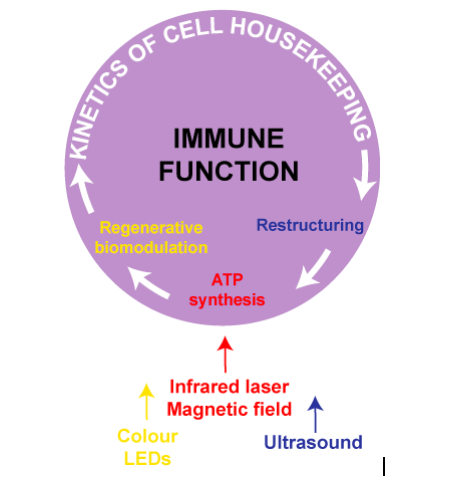
The bioenergetics of COVID-19 immunopathology and the therapeutic potential of biophysical radiances
1. Introduction
In a search for effective means of treating COVID-19, there are calls to investigate the biophysical effects of low intensity radiances, such as light [1,2]. In support of this quest, we ask a question - what is the exact nature of photobiomodulation in relation to the immune function of the human body? Some reports suggest enhanced function of immune cells: increased ability of human lymphocytes to bind Salmonella [3], increased proliferative potential of T-lymphocytes [4,5,6,7], enhanced cutaneous immune response to bacterial infection [8]. At the same time, numerous studies have shown reduced tissue inflammation and fibrosis [9,10,11]. Also, can other noninvasive radiances, such as magnetic field and ultrasound, play a role in enhancing immune response?
Furthermore, the diversity of biological effects of the radiances needs to be reconciled with the remarkably different trajectories of SARS-CoV-2 infections in humans: from completely asymptomatic to a severe course of disease. In the majority of infected individuals an adequate and timely immune response leads to the successful elimination of SARS-CoV-2 infection from the organism with mild pneumonia and sometimes asymptomatically [12,13]. Following antigen processing and presentation, B- and T-lymphocytes initiate the necessary responses of humoral and cellular immunity. In particular, within the first week after infection there is a rapid increase of antigen-specific cytotoxic T-lymphocytes that cause lysis of infected cells followed by resolution of symptoms [14].
However, in some infected individuals progressive dysfunction of the adaptive immune response has preceded more severe stages of the disease [15,16,17]. Published clinical data suggest the following chronological sequence:
- Individuals with preexisting circulatory and/or endocrine diseases were at a much greater risk of developing poorer clinical outcomes than those without comorbid conditions [18].
- After infection, T-lymphocytes show increasing signs of functional exhaustion. Expression of PD-1, TIM-3, CTLA-4, and TIGIT markers was at a minimum in the asymptomatic period, markedly increased in the symptomatic period and reached maximum levels while in Intensive Care Unit [15,19,20].
- If by days 10–12 after appearance of symptoms the proportion of lymphocytes drops below 5–15% and does not increase above 5% during the following week then the condition becomes critical, with very high risk of mortality [21,22].
- Overall, using only biomarkers of lactic dehydrogenase and lymphopenia it is possible to predict the severity and outcome of the disease more than 10 days in advance with more than 90% accuracy [23]. Remarkably, the prognostic horizon was estimated to be 18 days.
Based on this evidence a number of researchers have proposed that the severity of COVID-19 is driven by functional exhaustion and a decrease in the number of lymphocytes [15,16,24]. But what is the cause of such dysfunction of adaptive immunity? To our knowledge there is no explanation for the nature of the “switch” that, early in the disease, sets the trajectory to recovery or to a severe, critical course of the disease. This lack of understanding is a major obstacle towards development of an effective therapeutic modality for COVID-19 (Fig. 1).
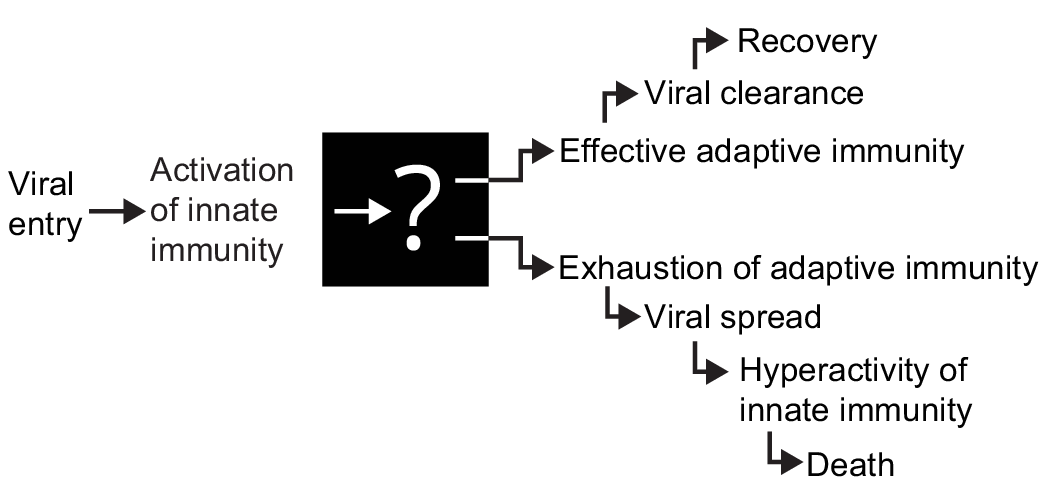
Fig. 1. After viral entry through the respiratory tract, early signs of innate immunity activation, such as cough, fever, fatigue are common in COVID-19. However, after the stage of activation of adaptive immunity there is a dramatic difference in severity and outcome of the disease.
In this theoretical paper we, firstly, place COVID-19 immunopathology within a unifying bioenergetic framework to demonstrate the biophysical underpinnings of immune function. Next, we propose that specific cell functions, such as immune function, depend on the kinetics of basal housekeeping of cells. And finally, we suggest that a comprehensive biophysical support using light, magnetic field and ultrasound may have a universal role in supporting cell function in immune defences and tissue repair.
2. Energy Depletion Underlies Immune Exhaustion in COVID-19
Since not everybody infected with the SARS-CoV-2 virus gets severely ill, the outcome of the disease depends not only on pathogen virulence but also on how effective are the defences of the host organism [25]. We propose that a study of the bioenergetics of immune function can explain the diversity of individual clinical outcomes of the disease and can create new opportunities for prevention and treatment of the disease. We present our hypothesis that the cause of immune exhaustion in COVID-19 and the subsequent worsening of a patient’s state is the result of energy deficit in immune cells that develops when a rapid increase of immune-related energetic expenditure is not met with sufficient functional reserve of the organism.
It is a well-established fact that immune function is a highly energetically-expensive process. Therefore, in order to spare energy, the inactive immune system does not function at 100% capacity and spends energy mostly on housekeeping of a limited population of immune cells [26]. But on the first encounter with a new pathogen, an individual’s immune system dramatically increases its energy requirements. Activation of innate immunity involves induction of fever at a cost of more than 10% increase in metabolic rate per 1° C rise in body temperature [27]. Next, activation of adaptive immunity leads to rapid and extensive cell growth and proliferation of virus-specific T- and B-lymphocytes that intensify energy metabolism in these cells by orders of magnitude [28]. Immune cells also require energy for performing various specialised effector functions, such as migration, phagocytosis and so on [26,29]. Altogether, while the total energy cost of the entire immune system in an inactive state is about 20% of an average daily resting metabolic rate in a healthy adult, inflammation may induce an increase of immune-related energy expenditure up to 25–60% (mild inflammation to sepsis) [26].
On other hand, experiments have shown that insufficient energy supply strongly limits adaptive immune function during the initial activation of T-lymphocytes and later during their effector activities [30]. Low supply of energy-rich macromolecules of glucose inhibits cell proliferation and proper functioning of T-lymphocytes, such as the production of cytokines. Finally, critical intracellular energy deficit leads to the death of immune cells.
The rapid increase in energy requirements of an activated immune system poses a major challenge to the whole organism. Under conditions of limited total energy supply, the body readjusts the interaction between all physiological systems in order to conserve energy. Daytime fatigue, loss of appetite, insulin resistance, increased blood coagulation, high blood pressure, muscle wasting are thought to be positively selected mechanisms of maximizing immune function at the expense of other systems [26]. The crucial implication of such systemic responses to infection is that the overall efficiency of the host’s defences and, therefore, survival in severe infection depends not only on the inherent properties of the immune system and the pathogen, but also on the functional reserve (tolerance limits) of the whole organism [31]. By functional reserve we mean the extent to which the function of a cell, organ or whole organism can be intensified in response to a stressor without going into pathological dysfunction.
Generally speaking, there are four closely interrelated aspects of functional reserve of the body [32]:
- Bioenergetic functional reserve is the degree by how much bioenergetics and other metabolic processes can be increased and sustained in order to meet not only the basal metabolic needs, but also the extra energetic cost of the activated immune system;
- Structural functional reserve depends on synthesis of structural and enzymatic proteins and the dynamic restructuring of tissue to adapt to new functional requirements;
- Regulatory functional reserve depends on the neuro-immuno-endocrine regulation that is responsible for redistribution of limited systemic resources in the most energy efficient manner;
- Psychological functional reserve is centred around behaviour, emotional and social adaptation, which are related to cognition, memory, will and motivation. Psychological functional reserve is to a large degree formed by the knowledge, practical skills and overall self-sufficiency of a person.
Of these four aspects, energy acts as the common denominator of the efficiency of immune response and age, presence of comorbid conditions, psychological stress, lack of sleep and rest and other negative factors. Let us place the two trajectories shown in Fig. 1 into this bioenergetics context.
In a relatively healthy individual the extra energy expenditure induced by the immune response to a SARS-CoV-2 infection could be well within the functional reserve of the body. The cost of activation of innate and adaptive immunity is fully compensated by limiting voluntary activity. The individual may not register any symptoms, even though more than half of asymptomatic patients had signs of pneumonia on the chest CT [13] (Fig. 2A).
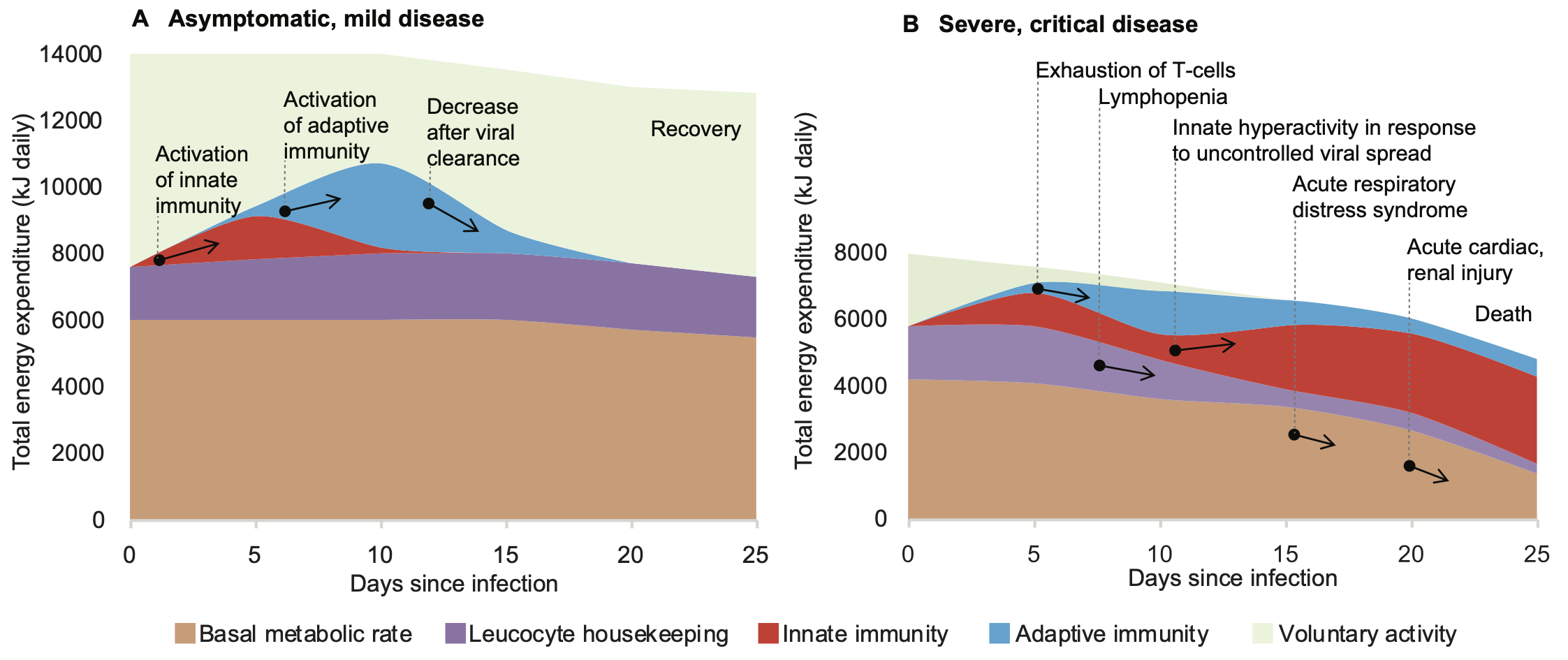
Fig. 2. The significant energy requirement of an activated immune response explains why in individuals with reduced functional reserve of the body adaptive immunity fails to contain infection. The aging process, circulatory diseases, diabetes and many other chronic conditions may reduce the ability of the body to reallocate energy towards immunity. The bioenergetics calculations are from [26,33] and the clinical course of COVID-19 is from [34].
However, if a person has one or more pre-existing chronic conditions, activation of the immune response could be limited by reduced functional reserve of the body during the first week of the disease (Fig. 2B). Overpowering fatigue could be an early sign of an insufficient energy supply that cannot be compensated by merely stopping voluntary activities. This deficit may develop as a result of reduced cardiovascular function that failed to elevate sufficiently transport of nutrients and oxygen, thus blocking the intensification of intracellular energy metabolism. Furthermore, impaired glucose metabolism in diabetes may prevent neuroendocrine redistribution of energy towards the immune function. Other chronic conditions can also create additional energetic burdens. For example, a chronic low-grade infection, such as Hepatitis C with fully compensated liver disease, increases energy expenditure by about 10% [35]. Age is also associated with a general decrease in metabolic rates, thus explaining higher mortality in the elderly. In situations of immediate danger (real or imaginary) the central nervous system also can have profound immunosuppressive effects via bioenergetic limitation. Psychomotor activity caused by acute psychological stress, sleep disturbance, pain, anxiety may create additional energetic expenditure up to 30% of basal metabolic rate [26]. Constant fear of getting infected by an “invisible virus”, feeling helpless and isolated in conditions of pandemia can also contribute towards immune dysregulation [36].
Overall, the sum total of energetic, structural, regulatory and psychological negative factors may critically limit the ability of the immune system to clear out the infection. Progressive energetic depletion leads to functional exhaustion of immune cells and, ultimately, to cell death (lymphopenia). Similar deterioration of immune function is also characteristic in chronic infections, autoimmune conditions and oncological diseases, but this occurs over much longer periods [37]. In COVID-19 the failure of adaptive immunity to contain infection rapidly leads to an uncontrolled viral spread that provokes secondary pathological hyperactivation of innate immunity (cytokine storm), acute respiratory distress syndrome, acute injury to the heart, kidneys, other organs and, finally, death.
What are the consequences of the bioenergetic hypothesis of COVID-19 immunopathology? First of all, direct methods of immunocorrection with medication (stimulation, suppression) may not succeed because they do not address the core issue of cellular energy deficit that limits immune function and even threatens cell survival (lymphopenia). Secondly, therapeutic options of oxygen and nutritive support are important, especially in critical conditions, but they are still not resolving the basic limitations of bioenergetic metabolism in a cell.
3. Biophysical Support for Basal Regeneration of a Cell
The bioenergetics hypothesis of COVID-19 highlights the fact that even though a number of different stressors may lead to cellular energy deficit and may manifest in an even wider variety of clinical signs and symptoms, a cell operates as one integrated whole. We propose that there is a set of metabolic processes of interconversions of energy, structure and information that continually recreate basal integrity of a cell. The kinetics of that basal metabolism, in turn, determines the ability of a cell to perform various specific functions.
To use an analogy, a person may ride a bicycle for leisure, sports or to transport heavy objects. While sitting on the bicycle, the person’s stability is inherently out of equilibrium and so the rider has to keep pedalling. Therefore, kinetic stability depends on continuous forward momentum, the kinetics of rotating wheels. By keeping the bicycle well lubricated and replacing worn out parts, the person may use it as a very efficient means of transportation for a long time.
Like a person riding a bicycle for different purposes, an immune cell may exist in an inactive state or engage in various immune activities (Fig. 3). But at all times the cell is far from being in a thermodynamic equilibrium and has to continuously regenerate itself to compensate for ongoing disintegration and to be able to do any biological work. From the point of view of thermodynamics, this re-assembly of a highly ordered structure and coherent function involves the reduction of entropy [38]. Entropy is a property that describes the number of degrees of freedom or, in other words, a state of order or disorder in a system. According to the second law of thermodynamics, a closed system tends to increase its entropy. Atoms in such a system do not assemble into complex structures or move in coherent ways but rather tend to dissipate and oscillate randomly, thus gaining more and more degrees of freedom. But many processes in a cell, such as the biosynthesis of macromolecules or the creation of concentration gradients, reduce the number of degrees of freedom and thus require energy. Consequently, a living cell is a thermodynamically open system that extracts energy and matter from the environment to maintain low internal entropy.
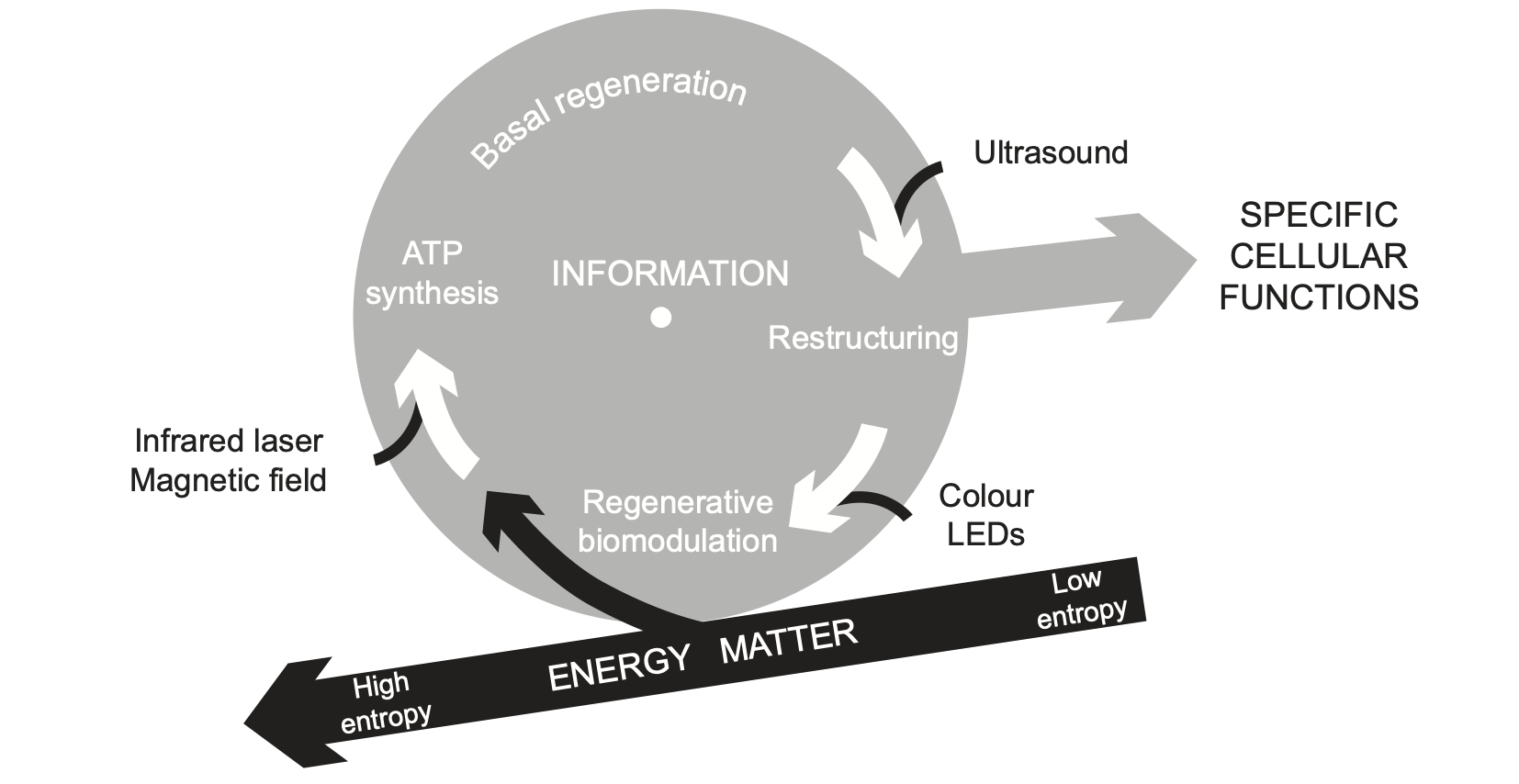
Fig. 3. A cell extracts energy and matter from the environment to decrease entropy locally, in spite of a global trend to a higher entropy. The generated order can be converted into biological work in the form of specific cellular functions, (immune, respiratory, etc) as well as adaptation and injury repair. The functional reserve of a cell depends on the kinetics of basal regeneration – rate of conversion of absorbed energy and matter into cellular structure and activity. We identified three metabolic processes that can be enhanced using biophysical stimuli: photomagnetic catalysis of ATP synthesis, regenerative biomodulation using visible monochromatic light and the ultrasonic acceleration of cell restructuring.
Unlike in a bicycle, all information necessary for self-regeneration is stored within a cell and not in the absorbed energy and matter [39]. A cell self-regulates the balance between disintegration and re-assembly to meet precisely the demands of activity and rest. Therefore, a cell is a thermodynamically open but operationally closed system [40]. Autonomy and self-sufficiency enable a cell and the whole human body to maintain their integrity through molecular, cellular and tissue regeneration, albeit within certain limits. The pool of ATP molecules in a cell has to be regenerated nearly every minute. Structural macromolecules are also replaced, although at a slower speed. A cardiomyocyte, for example, replaces nearly all its molecules in about two weeks [41]. Most cells in a human body are replaced at a rate of a few days to several decades [42]. Importantly, the same regenerative process enables cells and the human body not only to maintain integrity but also to adapt and self-repair.
The kinetic stability requirement for a cell’s existence and resilience to stress [43] creates a technological opportunity to support any cell function. One can increase the rate of a cell’s self-regeneration by enhancing certain low level transformations of energy, structure and signals through noninvasive biophysical stimuli. Importantly, such biophysical stimuli should not contradict each other and should also be of a low intensity to avoid disruption of cell structure and interference with cell regulation.
3.1. Biophysical Support for Basal Regeneration of a Cell
Biological work requires energy. For example, the activation, migration, antigen processing and presentation and the phagocytosis involved in immune function are energy-dependent [33]. To sustain these activities, a cell absorbs energy from the environment in bulk form, such as glucose and then converts it into a usable form through synthesis of ATP. However, the thermodynamic efficiency of this conversion via oxidative phosphorylation is estimated to be only 41% [44]. Such low conversion efficiency could be a major limiting factor in cell metabolism.
Since the early 1980s numerous studies have shown increased intracellular ATP synthesis after irradiation with low level infrared laser. Enhanced energetic metabolism has been observed in cells and tissue under conditions of ischemia, intoxication and intense physical exercises by up to 200–300% [45,46,47,48,49,50,51,52,53].
How can light increase ATP production in a mammalian cell that lacks photosynthetic apparatus? A number of light induced changes have been documented in cell bioenergetics [54,55], but the key question remains about the exact nature of the transformation of external electromagnetic stimuli into an increase in cellular chemical energy supply. In our opinion, the discovery of an additional radical pair reaction channel in ATP synthesis provides the most plausible explanation (Fig. 4 ) [56,57]. It is also possible that the proposed radical pair channel co-exists with other pathways such as photobiomodulation of water structure and function.
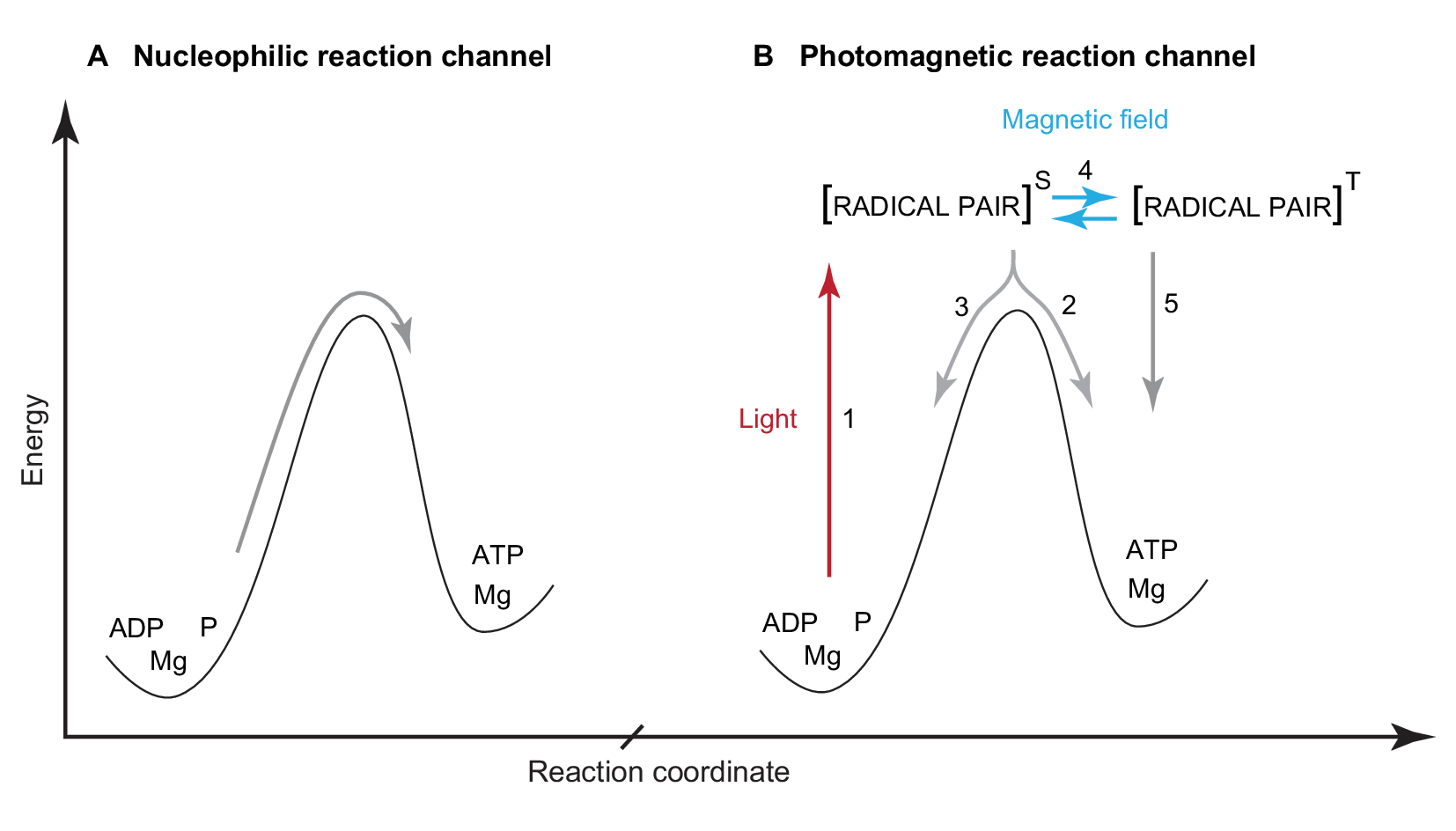
Fig. 4. In the generally-accepted nucleophilic channel of enzymatic ATP synthesis (A), the two molecules ADP and P are mechanically compressed to overcome the powerful repulsion between the negatively charged electron clouds of the two reactants. There is, however, an additional radical pair reaction channel (B) which is nearly an order of magnitude cheaper in energy than the nucleophilic compression [56]. Photoexcitation (1) shifts the reaction into forming a highly reactive intermediate state of a radical pair (radical-cation Mg+ and oxyradical of ADP) in singlet (S) spin state due to spin conservation during electron transfer. Next, phosphorylation leads to formation of ATP (2) but this process is supressed by spin-allowed electron back transfer and regeneration of the initial reactants (3). External magnetic field stimulates conversion (4) of the radical pair into a triplet state (T) in which electron back transfer is spin-forbidden thus increasing the total ATP production (5).
In the catalytic site of ATP producing enzyme inorganic phosphate is added to ADP. Both light and magnetic field can increase the yield of ATP synthesis by opening an additional radical pair reaction channel. Analysis of the kinetics of the high-energy radical pair channel and the low-energy nucleophilic channel have shown that the efficiency of the latter is only 8%. Only one molecule of ATP is formed from 12 pairs of ADP and P molecules sequentially entering into the catalytic site of an ATP-producing enzyme. The remaining 11 pairs of ADP and P revert to their original disjointed state. On the other hand, the radical pair channel is twice as efficient. Furthermore, the radical pair channel explains synergy of action between light and magnetic field in the enhancement of ATP synthesis, DNA synthesis [58] and light-dependent magnetoreception in birds [59]. Once a radical pair is formed through photoexcitation, thermodynamic control (activation barrier) no longer applies, but there is also kinetic control through a strict limitation on the spin coherence of unpaired electrons. In the presence of an external magnetic field the spin of the radical pair interconverts between singlet and triplet states and thus favours towards ATP formation rather than reverting to the initial reactants [60,61].
To summarize, one can create conditions of 200–300% enhanced ATP synthesis artificially through the combined application of low level infrared light and weak magnetic field. Friedmann et al. measured the synergistic effect of these two radiances on electron transfer rates in the respiratory chain in the mitochondria, which is directly related to the rate of ATP synthesis [62]. The combination of these low intensity radiances produced a 22% change in electron paramagnetic resonance (EPR) signal. However, when the radiances were applied individually, they produced no measurable EPR signal. In fact, in the absence of a magnetic field, it took a 10-fold increase in light intensity before an 18% change in EPR signal could be reached. The experiment shows that by combining infrared laser and a weak magnetic field we can decrease the intensity of the individual radiances, while achieving a better biological effect.
The increased efficiency of ATP synthesis implies more available energy for cell function, even under unfavourable conditions of oxygen/glucose deficiencies or increased functional load. This biophysical effect of photomagnetic catalysis would explain the most diverse effects of Low Level Laser Therapy (LLLT) on enhanced regeneration of the heart, bone, brain, skin and other tissues [63], increased sports performance [64] and the number of studies in Russia that have suggested the efficacy of Magnetic Infrared Laser Therapy (MILT) for the treatment of a wide variety of conditions over the past 40 years [65].
In the area of immunology, application of LLLT/MILT has improved the functional reserve of immune cells: increased the ability of human lymphocytes to bind Salmonella [3], increased the proliferative potential of T-lymphocytes [4,5,6,7] and enhanced cutaneous immune response to bacterial infection [8]. Improved immune function has also been observed in clinical studies of treating respiratory conditions with immunodeficiencies of different origins:
- Up to 3-fold increase of suppressed functional reserve of neutrophils in patients with community-acquired pneumonia (Fig. 5 ) [8];
- Increased proliferation and functional activity of T-lymphocytes in patients with severe pneumonia [66];
- Increased proliferation of T-lymphocytes, increased IgA and IgG in paediatric patients with secondary immunodeficiency due to cystic fibrosis [67];
- Increased proliferation and functional activity of T- and B-lymphocytes in lung cancer patients [68].
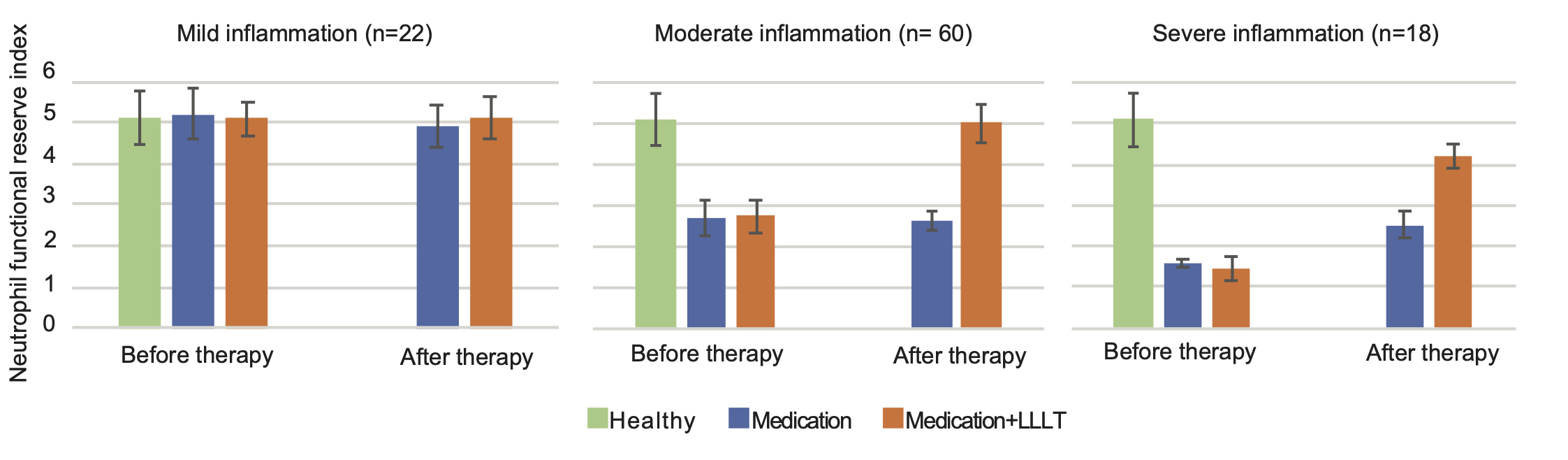
Fig. 5. Changes in neutrophil functional reserve after 7-day treatment course with medication (blue) and medication combined with LLLT (orange) in patients with community-acquired pneumonia [data from 8]. Functional reserve of healthy individuals (n = 30) is shown in green for comparison. The functional reserve index of neutrophils was estimated from their ability to reduce nitroblue tetrazolium in both spontaneous and stimulated tests. (For interpretation of the references to colour in this figure legend, the reader is referred to the web version of this article.)
There are also reports that LLLT/MILT reduce autoimmune injury: inhibition of LPS-induced neutrophilic lung inflammation and pro-inflammatory mediators in animal models of lung injury [11,69]; correction of imbalances between pro-inflammatory and anti-inflammatory cytokines in patients with pleural effusions of various aetiologies [70]; significant reduction of cytokinemia, faster elimination of clinical symptoms such as cessation of bacterial excretion, closure of decay cavities and decrease in hospital stay of patients with infiltrative pulmonary tuberculosis [71]. These therapeutic effects are especially important in COVID-19 because of the hyperinflammation damage during the later stages of the disease.
In our opinion there is no contradiction between the reports of enhanced immune response and of reduction of inflammation-related injury after LLLT/MILT. The primary biophysical effect of enhanced efficiency of ATP production increases the overall functional reserve of immune cells instead of directly increasing specific pro-inflammatory or anti-inflammatory processes. Therefore, improved pathogen elimination in the early stage of infection after LLLT/MILT would naturally lead to a faster decrease of pro-inflammatory signals in the later stages. Overall, one can expect that a more rapid and effective immune response would result in a shorter duration of inflammation, less immune-related tissue damage and a shorter recovery time.
However, there is also an important issue of selecting optimal parameters of laser used for LLLT. When a laser is used at too low intensity (irradiance), it may not produce sufficient therapeutic benefit, while too high intensity may lead to inhibitory side-effects effects expressed as cellular damage and increased pro-inflammatory response.
3.2. Accelerating Cell Restructuring with Low Intensity Ultrasound
Cell housekeeping and function also involve dynamic restructuring [72]. This process is most intensive during cell movements when a cell changes its shape in order to migrate, or to bring in or out particles. These movements are possible through the rapid regeneration of a cell’s internal scaffolding, as actin filaments are degraded and rebuilt into a remodelled cytoskeleton. In general, fast cell restructuring is essential for cell and tissue repair, immune function, formation of neural networks, communication and so on.
Ultrasound is an acoustical pressure wave (>20 kHz) that creates alternating zones of high and low pressure as it travels through media. In irradiated tissue, radiative acoustic force causes nanoscale oscillation of particles resulting in fluid motion, enhanced molecular transport and enzymatic lysis [73,74,75]. While high intensity ultrasound may excessively disrupt the cell membrane and cytoskeleton, low intensity ultrasound almost immediately increases the speed of actin restructuring [76,77] and enhances cell motility [78], phagocytosis [79] while increasing overall cell viability and metabolism [80,81,82]. These beneficial effects may explain how low intensity pulsed ultrasound (LIPUS) improves the healing of injured bones, tendons, cartilage, dental tissue, diabetic ulcers and neurological conditions [83,84,85].
Interestingly, LLLT/MILT and LIPUS may complement each other in a regenerative process, since the former provides energy for biosynthesis and the latter enhances controlled enzymatic degradation. Several studies have shown the synergism of these modalities in the treatment of bone fractures [86], hand osteoarthritis [87] and knee osteoarthritis [88,89]. In patients with chronic obstructive pulmonary disease, the sequential application of LLLT and LIPUS was found to be effective in stimulating excretion of sputum, reduction of inflammatory markers, improvement of external respiratory function and the general state of the patients [90].
3.3. Photobiomodulation Using Visible Monochromatic Light
Regulation of biological processes in a rhythmical pattern is crucial for long term survival of any organism. A basic physiological cycle consists of an active phase, when stored energy is converted into biological work and a rest phase that is dominated by processes of biosynthesis and recovery. For example, practically all physiological processes in the human body follow the daylight cue to switch between a mostly catabolic phase during the day and a mostly anabolic phase during the night. However, failure of regulatory systems to provide rest/activity rhythms may lead to eventual exhaustion and pathological changes. Chronic exposure to only one type of signal, such as immune activation in response to a persistent pathogen, may lead to maladaptative chronic inflammation and functional exhaustion of immune cells [26,91].
Experimental evidence suggests that visible monochromatic light has distinct modulatory effects on biological activity of cells and organisms through pathways that are possibly different from those of infrared laser light on cellular bioenergetics (Section 2.1). In vitro experiments have shown that, generally speaking, the blue end of the visible spectrum has an inhibitory effect, while light at the red end has an excitatory effect on cell metabolism, proliferation and differentiation in human dermal fibroblasts [92] and human adipose-derived stem cells [93,94]. Regeneration of planarian flatworms also have shown a similar response pattern to visible light of different wavelengths (colours) [95].
Interestingly, the ability of monochromatic light to correct imbalances in the state of regulatory systems has been used therapeutically for centuries. The Persian physician, Ibn Sina (Avicenna), built a treatment alcove where patients were exposed to sunlight filtered through different coloured glass. In his treatise Canon of Medicine, written in 1025, he described the stimulatory effect of the red colour on blood circulation and the “cooling” effect of the blue colour. During the 19th and 20th centuries a number of therapies were developed based on the phenomenon of colour phototherapy [96]. For example, in the 1920s Spitler pioneered syntonic optometry: the application of colour light directly to the eyes to treat visual dysfunctions, head trauma, headaches and problems with learning and behaviour [97,98]. He collated his experiences with more than 3000 patients and formulated a set of guidelines that prescribe certain colour filters to normalise (syntonise) emotional, mental and visual disorders. Red and orange colours stimulate the sympathetic nervous system (‘fight or flight’ response). Indigo and blue colours activate the parasympathetic nervous system (‘rest and digest’ response). In the mid-section between these two opposites, the yellow-green colour promotes physiological balance. Pupil and visual field testing allow objective measurements of the therapeutic progress.
4. Coherent Synthesis of Biophysical Radiances
Basal housekeeping of a cell is a unity of processes composed of interactions of information, energy and matter. Each of these fundamental categories is equally important to cell survival and function [99]. This interdependence suggests that there is a potential synergy between the biophysical effects of low level infrared laser, magnetic field, colour LEDs and ultrasound. Therefore, our discussion of enhancing specific cell functions via biophysically improved kinetics of basal metabolism (Section 2) would be incomplete without considering the synthesis of these radiances.
The scientific principle of coherent multi-radiances has been implemented in a full-scale invention of a new medical technology - coMra™ therapy [100]. CoMra devices coherently synthesize all of the above-mentioned biophysical radiances into a single therapeutic stream. Coherency of such synthesis is achieved via the geometric arrangement of radiance emitters, matched output power of each emitter and harmonic modulation of all emitters except for the permanent magnetic field (Fig. 6).
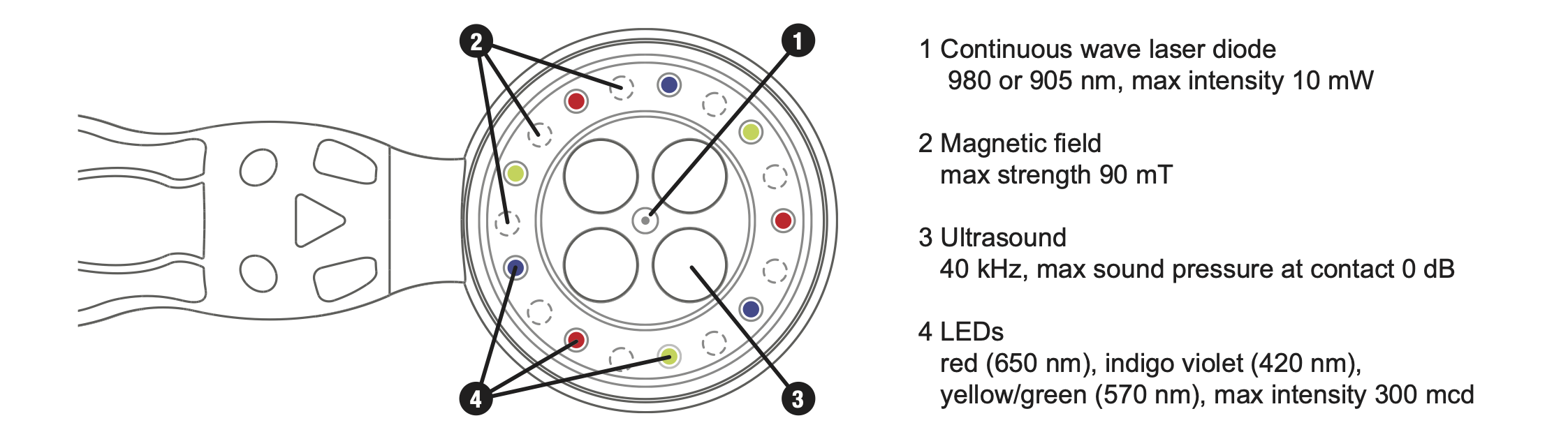
Fig. 6. Bottom view of the medical terminal of the coMra Delta device.
Furthermore, since each radiance synergistically supports the same process of regeneration, the energy output of the individual emitters in coMra devices is much lower in comparison to monotherapy devices. This ensures safety, as well as simplicity of application of coMra devices, while aiming at maximum therapeutic efficacy. In the context of the COVID-19 pandemia this is especially important, since coMra devices can readily be used both in a medical facility and at home during self-isolation.
And most importantly, these design features of coMra devices create an opportunity for transforming a passive recipient of complex and expensive medical procedures into an active builder of one’s own health. As described by Antonovsky, a major determinant of either succumbing to a disease or staying healthy is a psychological sense of coherence, an “enduring though dynamic feeling of confidence” that one has the necessary resources to meet life’s challenges in a meaningful way [101]. To this end, coMra devices were designed to be used not only under the supervision of a doctor, but primarily as a self-care therapeutic modality that can readily complement one’s lifestyle choices.
4.1. Regenerative Biomodulation Via Sequenced Colour LEDs
coMra therapy also introduced a concept of regenerative biomodulation via sequenced colour LEDs. This concept arises from a proposal that to form a regenerative rhythm regulatory signals should follow a sequence of three states. The first signal is upregulation of a biological process from a basal level. The second signal is downregulation that inhibits the ongoing process to avoid exhaustion and energy is instead redirected towards the recovery of spent resources. The third distinct type of signal is to resume the most energy saving configuration of relative inactivity at a (new) basal level. Together, when the three signals are repeated in succession, they form a composite regenerative signal.
We suggest that this is a biologically universal signal that spans regulation of individual cells and the entire human body. Immune and nervous systems provide indirect evidence of the existence of such regulation. During normal wound healing the immune response starts with 1) proinflammatory signals to activate immune response. Then, onset of 2) the anti-inflammatory phase stimulates cell proliferation. Finally, during 3) the remodelling phase a balance between proinflammatory and anti-inflammatory factors is required to replace temporary tissue with a permanent one [102]. Similarly, the human autonomic nervous system has evolved into three distinct systems: 1) mobilization via the sympathetic system, 2) parasympathetic inhibition via myelinated vagus, 3) immobilization via unmyelinated vagus [103].
In coMra devices this triple regenerative signal is created by a sequence of red colour (650 nm), indigo/violet colour (420 nm) and a combination of yellow and green colours (570 nm) and is delivered through dermal photobiomodulation [104] (Fig. 7).
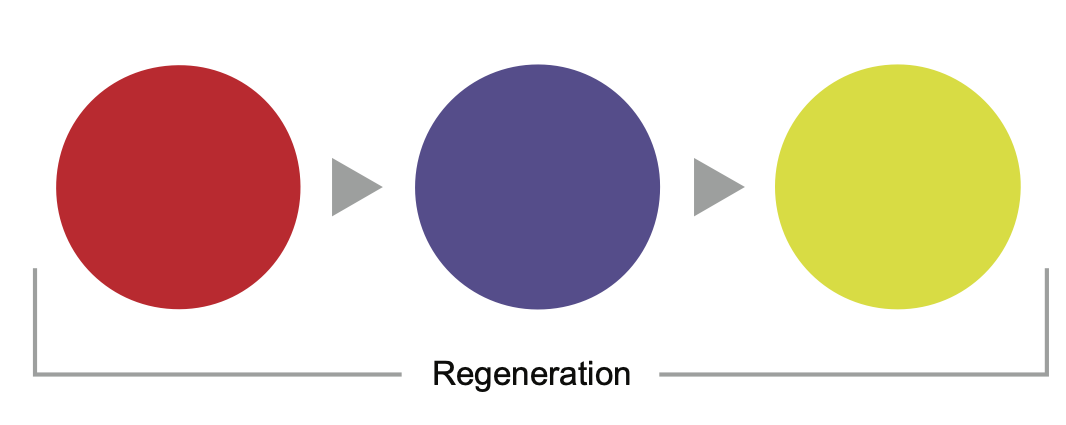
Fig. 7. Sequence of colour LEDs used in coMra therapy to provide a composite regenerative signal.
5. Conclusion
The proposed biophysical paradigm of COVID-19 immunopathology and treatment reconciles the diverse trajectories of SARS-CoV-2 infections and also unifies various effects of low level biophysical radiances on immune function. We suggested that interconversions of energy, structure and information involved in regeneration of a cell are the key prerequisite for any cell function. Specific immune functions of a cell, on the other hand, are always context-dependent and are activated by the organism’s response to a pathogen up to the limit imposed by the kinetics of self-regeneration.
To enhance the immune function of a cell and of the human body, one can target the basal regeneration via biophysical means: photomagnetic catalysis of ATP synthesis, ultrasonic acceleration of cytoskeleton restructuring and introduction of regenerative biomodulation via sequenced colour LEDs. Importantly, the biophysically enhanced cell regeneration presupposes neither a specific cell function nor type of a pathogen. Therefore, we envision application of the described biophysical therapies for the treatment of immunodeficient conditions and any current or future viral infections. Functional exhaustion of lymphocytes and lymphopenia are characteristic not only of COVID-19, but were also observed in the SARS-CoV outbreak in 2003, H1N1 in 2009, MERS-CoV in 2013 [105]. Finally, for those individuals who are recovering after COVID-19, the biophysical modalities may offer significant benefits in terms of regeneration of organs and systems that have sustained inflammation-related damage.
References
- Enwemeka C.S. Light as a potential treatment for pandemic coronavirus infections: a perspective. J. Photochem. Photobiol. B Biol. 2020;111891(207) doi: 10.1016/j.jphotobiol.2020.111891.
- Fekrazad R. Photobiomodulation and antiviral photodynamic therapy as a possible novel approach in COVID-19 management. Photobiomodulation, Photomed. Laser Surg. 2020 doi: 10.1089/photob.2020.4868.
- Passarella S. Quantitative analysis of lymphocyte-Salmonella interaction and effect of lymphocyte irradiation by helium-neon laser. Biochem. Biophys. Res. Commun. 1985;130(2):546–552. doi: 10.1016/0006-291X(85)90451-6.
- Agaiby A.D. Laser modulation of angiogenic factor production by T-lymphocytes. Lasers Surg. Med. 2000;26(4):357–363. doi: 10.1002/(SICI)1096-9101(2000)26:4%3C357::AID-LSM3%3E3.0.CO;2-O.
- Gulsoy M. The biological effects of 632.8-nm low energy he–ne laser on peripheral blood mononuclear cells in vitro. J. Photochem. Photobiol. B Biol. 2006;82(3):199–202. doi: 10.1016/j.jphotobiol.2005.11.004.
- Stadler I. In vitro effects of low-level laser irradiation at 660 nm on peripheral blood lymphocytes. Lasers Surg. Med. 2000;27(3):255–261. doi: 10.1002/1096-9101(2000)27:3<255::aid-lsm7>3.0.co;2-l.
- Wasik M. The influence of low-power helium-neon laser irradiation on function of selected peripheral blood cells. J. Physiol. Pharmacol. 2007;58(Pt 2):729–737.
- Burduli N.M., Gabueva A.A. The influence of low-intensity laser radiation on the functional activity of neutrophils in the patients presenting with community-acquired pneumonia. Vopr. Kurortol. Fizioter. Lech. Fiz. Kult. 2016;93(2):9–12. 10.17116/kurort201629-12 [in Russian]
- Walski T. The effect of red-to-near-infrared (R/NIR) irradiation on inflammatory processes. Int. J. Radiat. Biol. 2019;95(9):1326–1336. doi: 10.1080/09553002.2019.1625464.
- de Lima F.M. Low-level laser therapy associated to N-acetylcysteine lowers macrophage inflammatory protein-2 (MIP-2) mRNA expression and generation of intracellular reactive oxygen species in alveolar macrophages. Photomed. Laser Surg. 2010;28(6):763–771. doi: 10.1089/pho.2009.2638.
- Cury V. Low level laser therapy reduces acute lung inflammation without impairing lung function. J. Biophotonics. 2016;9(11−12):1199–1207. doi: 10.1002/jbio.201500113.
- Guan W.-J. Clinical characteristics of coronavirus disease 2019 in China. N. Engl. J. Med. 2020 doi: 10.1056/NEJMoa2002032.
- Kong W. Comparison of clinical and epidemiological characteristics of asymptomatic and symptomatic SARS-CoV-2 infection: a multi-center study in Sichuan Province, China. Travel Med. Infect. Dis. 2020;101754 doi: 10.1016/j.tmaid.2020.101754.
- Thevarajan I. Breadth of concomitant immune responses prior to patient recovery: a case report of non-severe COVID-19. Nat. Med. 2020;26(4):453–455. doi: 10.1038/s41591-020-0819-2.
- Diao B. Reduction and functional exhaustion of T cells in patients with coronavirus disease 2019 (COVID-19) Front. Immunol. 2020;11:827. doi: 10.3389/fimmu.2020.00827.
- Qin C. Dysregulation of immune response in patients with COVID-19 in Wuhan, China. Clin. Infect. Dis. 2020 doi: 10.1093/cid/ciaa248.
- Bo X. Suppressed T cell-mediated immunity in patients with COVID-19: a clinical retrospective study in Wuhan, China. J. Infect. 2020 doi: 10.1016/j.jinf.2020.04.012.
- Guan W.-J. Comorbidity and its impact on 1590 patients with COVID-19 in China: a nationwide analysis. Eur. Respir. J. 2020;55(5):2000547. doi: 10.1183/13993003.00547-2020.
- Zheng M. Functional exhaustion of antiviral lymphocytes in COVID-19 patients. Cell. Mol. Immunol. 2020 doi: 10.1038/s41423-020-0402-2.
- Zheng H.-Y. Elevated exhaustion levels and reduced functional diversity of T cells in peripheral blood may predict severe progression in COVID-19 patients. Cell. Mol. Immunol. 2020 doi: 10.1038/s41423-020-0401-3.
- Tan L. Lymphopenia predicts disease severity of COVID-19: a descriptive and predictive study. Signal Transduction Targeted Ther. 2020;5(1):33. doi: 10.1038/s41392-020-0148-4.
- Giamarellos-Bourboulis E.J. Complex immune dysregulation in COVID-19 patients with severe respiratory failure. Cell Host Microbe. 2020 doi: 10.1016/j.chom.2020.04.009.
- Yan L. An interpretable mortality prediction model for COVID-19 patients. Nat. Machine Intell. 2020 doi: 10.1038/s42256-020-0180-7.
- Lin L. Hypothesis for potential pathogenesis of SARS-CoV-2 infection - a review of immune changes in patients with viral pneumonia. Emerging Microb. Infect. 2020 doi: 10.1080/22221751.2020.1746199.
- Ayres J.S. The biology of physiological health. Cell. 2020;181(2):250–269. doi: 10.1016/j.cell.2020.03.036.
- Straub R.H. The brain and immune system prompt energy shortage in chronic inflammation and ageing. Nat. Rev. Rheumatol. 2017;13:743. doi: 10.1038/nrrheum.2017.172.
- Kluger M. Phylogeny of fever. Fed. Proc. 1979;38(1):30–34.
- MacIver N.J. Glucose metabolism in lymphocytes is a regulated process with significant effects on immune cell function and survival. J. Leukoc. Biol. 2008;84(4):949–957. doi: 10.1189/jlb.0108024.
- Straub R.H. Energy regulation and neuroendocrine–immune control in chronic inflammatory diseases. J. Intern. Med. 2010;267(6):543–560. doi: 10.1111/j.1365-2796.2010.02218.x.
- Jacobs S.R. Glucose uptake is limiting in T cell activation and requires CD28-mediated Akt-dependent and independent pathways. J. Immunol. 2008;180(7):4476–4486.
- Ayres J.S. Surviving COVID-19: a disease tolerance perspective. Sci. Adv. 2020 doi: 10.1126/sciadv.abc1518. eabc1518.
- Kurzanov A.N. Izdatelskiy dom Academii Estestovznaniy; Moscow: 2016. Functional Reserves of Organism. (in Russian)
- Buttgereit F. Bioenergetics of immune functions: fundamental and therapeutic aspects. Immunol. Today. 2000;21(4):194–199.
- Zhou F. Clinical course and risk factors for mortality of adult inpatients with COVID-19 in Wuhan, China: a retrospective cohort study. Lancet. 2020;395(10229):1054–1062. doi: 10.1016/S0140-6736(20)30566-3.
- Piche T. Resting energy expenditure in chronic hepatitis C. J. Hepatol. 2000;33(4):623–627. doi: 10.1016/S0168-8278(00)80014-8.
- Kiecolt-Glaser J.K. Emotions, morbidity, and mortality: new perspectives from psychoneuroimmunology. Annu. Rev. Psychol. 2002;53(1):83–107. doi: 10.1146/annurev.psych.53.100901.135217.
- McLane L.M. CD8 T cell exhaustion during chronic viral infection and cancer. Annu. Rev. Immunol. 2019;37(1):457–495. doi: 10.1146/annurev-immunol-041015-055318.
- Davies P.C.W. Self-organization and entropy reduction in a living cell. Bio Systems. 2013;111(1):1–10. doi: 10.1016/j.biosystems.2012.10.005.
- Maturana H.R., Varela F.J. Vol. 42. Springer Science & Business Media; 1980. Autopoiesis and cognition: the realization of the living.
- Capra F., Luisi P.L. Cambridge University Press; 2014. The Systems View of Life: A Unifying Vision.
- Gevers W. Protein metabolism of the heart. J. Mol. Cell. Cardiol. 1984;16(1):3–32. doi: 10.1016/S0022-2828(84)80711-7.
- Milo R., Phillips R. Garland Science; 2015. Cell Biology by the Numbers.
- Pross A., Khodorkovsky V. Extending the concept of kinetic stability: toward a paradigm for life. J. Phys. Org. Chem. 2004;17(4):312–316. doi: 10.1002/poc.729.
- Nath S. The thermodynamic efficiency of ATP synthesis in oxidative phosphorylation. Biophys. Chem. 2016;219:69–74. doi: 10.1016/j.bpc.2016.10.002.
- Passarella S. Increase of proton electrochemical potential and ATP synthesis in rat liver mitochondria irradiated in vitro by helium-neon laser. FEBS Lett. 1984;175(1):95–99. doi: 10.1016/0014-5793(84)80577-3.
- Vekshin N.L. Light-dependent ATP synthesis in mitochondria. Biochem. Int. 1991;25(4):603–611.
- Karu T. Irradiation with HeNe laser increases ATP level in cells cultivated in vitro. J. Photochem. Photobiol. B Biol. 1995;27(3):219–223. doi: 10.1016/1011-1344(94)07078-3.
- Oron U. Ga-as (808 nm) laser irradiation enhances ATP production in human neuronal cells in culture. Photomed. Laser Surg. 2007;25(3):180–182. doi: 10.1089/pho.2007.2064.
- Benedicenti S. Intracellular ATP level increases in lymphocytes irradiated with infrared laser light of wavelength 904 nm. Photomed. Laser Surg. 2008;26(5):451–453. doi: 10.1089/pho.2007.2218.
- Oron U. Low-energy laser irradiation reduces formation of scar tissue after myocardial infarction in rats and dogs. Circulation. 2001;103(2):296–301. doi: 10.1161/01.CIR.103.2.296.
- Rojas J.C. Neuroprotective effects of near-infrared light in an in vivo model of mitochondrial optic neuropathy. J. Neurosci. 2008;28(50):13511–13521. doi: 10.1523/jneurosci.3457-08.2008.
- Lapchak P.A., De Taboada L. Transcranial near infrared laser treatment (NILT) increases cortical adenosine-5′-triphosphate (ATP) content following embolic strokes in rabbits. Brain Res. 2010;1306:100–105. doi: 10.1016/j.brainres.2009.10.022.
- Ferraresi C. Light-emitting diode therapy in exercise-trained mice increases muscle performance, cytochrome c oxidase activity, ATP and cell proliferation. J. Biophotonics. 2015;8(9):740–754. doi: 10.1002/jbio.201400087.
- Passarella S., Karu T. Absorption of monochromatic and narrow band radiation in the visible and near IR by both mitochondrial and non-mitochondrial photoacceptors results in photobiomodulation. J. Photochem. Photobiol. B Biol. 2014;140(0):344–358. doi: 10.1016/j.jphotobiol.2014.07.021.
- Castro K.M.R. Can photobiomodulation therapy (PBMT) control blood glucose levels and alter muscle glycogen synthesis? J. Photochem. Photobiol. B Biol. 2020;207:111877. doi: 10.1016/j.jphotobiol.2020.111877.
- Buchachenko A.L. Chemistry of enzymatic ATP synthesis: an insight through the isotope window. Chem. Rev. 2012;112(4):2042–2058. doi: 10.1021/cr200142a.
- Buchachenko A.L. A specific role of magnetic isotopes in biological and ecological systems. Physics and biophysics beyond. Prog. Biophys. Mol. Biol. 2020;155:1–19. doi: 10.1016/j.pbiomolbio.2020.02.007.
- Buchachenko A., Lawler R.G. New possibilities for magnetic control of chemical and biochemical reactions. Acc. Chem. Res. 2017;50(4):877–884. doi: 10.1021/acs.accounts.6b00608.
- Hore P.J., Mouritsen H. The radical-pair mechanism of magnetoreception. Annu. Rev. Biophys. 2016;45(1):299–344. doi: 10.1146/annurev-biophys-032116-094545.
- Buchachenko A. Coherent chemistry and biochemistry. Russ. J. Gen. Chem. 2011;81(1):170–180. doi: 10.1134/s1070363211010348.
- Ivanov K.L. Spin-chemistry concepts for spintronics scientists. Beilstein J. Nanotechnol. 2017;8(1):1427–1445. doi: 10.3762/bjnano.8.143.
- Friedmann H. Combined magnetic and pulsed laser fields produce synergistic acceleration of cellular electron transfer. Laser Ther. 2009;18(3):137–141. doi: 10.5978/islsm.18.137.
- Abrahamse H., Hamblin M.R. Morgan & Claypool Publishers; 2017. Photomedicine and Stem Cells.
- Ferraresi C. Photobiomodulation in human muscle tissue: an advantage in sports performance? J. Biophotonics. 2016;9(11–12):1273–1299. doi: 10.1002/jbio.201600176.
- Kartelishev A.V. Practical medicine; Moscow: 2012. Laser Therapy and Prophylaxis. (in Russian)
- Kovaleva L.I. Comparative analysis of different immunity correction techniques in patients with secondary pneumonia. Bull. Respir. Physiol. Pathol. 2002;12:37–39. (in Russian)
- Shadrina E.M. Immunotherapy of patients with mucoviscidosis by magnetic-infrared-laser therapy. Kuban Sci. Med. Herald. 2012;5:76–78. (in Russian)
- Sheiko E.A. Immunocorrection obtained with monochromatic red light from emitting diodes in lung cancer patients. Laser Med. 2010;14(2):49–52. (in Russian)
- Mafra de Lima F. Low intensity laser therapy (LILT) in vivo acts on the neutrophils recruitment and chemokines/cytokines levels in a model of acute pulmonary inflammation induced by aerosol of lipopolysaccharide from Escherichia coli in rat. J. Photochem. Photobiol. B Biol. 2010;101(3):271–278. doi: 10.1016/j.jphotobiol.2010.07.012.
- Batirova M.E. Dynamics of some parameters of the cytokine profile in exudative pleuritis of various etiology under intravenous laser blood irradiation (interim results) Laser Med. 2017;21(3):28–31. (in Russian)
- Sutyagina D.A. Complex therapy of infiltrative pulmonary tuberculosis with the use of low-intensity laser radiation: features of dynamics of cytokines, the treatment effectiveness. J. New Med. Technol. 2019;13(1) doi: 10.24411/2075-4094-2019-16229. [in Russian]
- Pollard T.D. Elsevier Health Sciences; 2016. Cell Biology.
- Veronick J. The effect of acoustic radiation force on osteoblasts in cell/hydrogel constructs for bone repair. Exp. Biol. Med. 2016;241(10):1149–1156.
- Suchkova V. Ultrasound enhancement of fibrinolysis at frequencies of 27 to 100 kHz. Ultrasound Med. Biol. 2002;28(3):377–382. doi: 10.1016/S0301-5629(01)00522-1.
- Chernysh I.N. Molecular mechanisms of the effect of ultrasound on the fibrinolysis of clots. J. Thromb. Haemost. 2015;13(4):601–609. doi: 10.1111/jth.12857.
- Samandari M. Ultrasound induced strain cytoskeleton rearrangement: an experimental and simulation study. J. Biomech. 2017;60:39–47.
- Mizrahi N. Low intensity ultrasound perturbs cytoskeleton dynamics. Soft Matter. 2012;8(8):2438–2443. doi: 10.1039/C2SM07246G.
- Atherton P. Low-intensity pulsed ultrasound promotes cell motility through vinculin-controlled Rac1 GTPase activity. J. Cell Sci. 2017;130(14):2277–2291. doi: 10.1242/jcs.192781.
- Zhou S. Low intensity pulsed ultrasound accelerates macrophage phagocytosis by a pathway that requires actin polymerization, rho, and Src/MAPKs activity. Cell. Signal. 2008;20(4):695–704. doi: 10.1016/j.cellsig.2007.12.005.
- Tsuang Y.-H. Effects of low intensity pulsed ultrasound on rat schwann cells metabolism. Artif. Organs. 2011;35(4):373–383. doi: 10.1111/j.1525-1594.2010.01086.x.
- Samuels J.A. Low-frequency (<100 kHz), low-intensity (<100 mW/cm(2)) ultrasound to treat venous ulcers: a human study and in vitro experiments. J. Acoustical Soc. Am. 2013;134(2):1541–1547. doi: 10.1121/1.4812875.
- Doan N. In vitro effects of therapeutic ultrasound on cell proliferation, protein synthesis, and cytokine production by human fibroblasts, osteoblasts, and monocytes. J. Oral Maxillofac. Surg. 1999;57(4):409–419. doi: 10.1016/S0278-2391(99)90281-1.
- Jiang X. A review of low-intensity pulsed ultrasound for therapeutic applications. IEEE Trans. Biomed. Eng. 2018;66(10):2704–2718. doi: 10.1109/TBME.2018.2889669.
- Escoffre J.-M., Bouakaz A. Springer; 2015. Therapeutic Ultrasound.
- Alexandrov A.V. Ultrasound-enhanced systemic thrombolysis for acute ischemic stroke. N. Engl. J. Med. 2004;351(21):2170–2178. doi: 10.1056/NEJMoa041175.
- Babuccu C. Cumulative effect of low-level laser therapy and low-intensity pulsed ultrasound on bone repair in rats. Int. J. Oral Maxillofac. Surg. 2014;43(6):769–776. doi: 10.1016/j.ijom.2013.12.002.
- Paolillo A. Synergic effects of ultrasound and laser on the pain relief in women with hand osteoarthritis. Lasers Med. Sci. 2015;30(1):279–286. doi: 10.1007/s10103-014-1659-4.
- Paolillo F.R. Ultrasound plus low-level laser therapy for knee osteoarthritis rehabilitation: a randomized, placebo-controlled trial. Rheumatol. Int. 2018;38(5):785–793. doi: 10.1007/s00296-018-4000-x.
- De Souza Simão M. Sinergic effect of therapeutic ultrasound and low-level laser therapy in the treatment of hands and knees ostheoarthritis. J. Arthrit. 2018;7(6) doi: 10.4172/2167-7921.1000277.
- Nikitin V.A. Laser-ultrasound therapy in the comprehensive treatment of chronic obstructive pulmonary disease. Tuber. Lung Dis. 2018;96(8):31–36. 10.21292/2075-1230-2018-96-8-31-36 [in Russian]
- Cohen S. Chronic stress, glucocorticoid receptor resistance, inflammation, and disease risk. Proc. Natl. Acad. Sci. 2012;109(16):5995–5999. doi: 10.1073/pnas.1118355109.
- Mignon C. Differential response of human dermal fibroblast subpopulations to visible and near-infrared light: potential of photobiomodulation for addressing cutaneous conditions. Lasers Surg. Med. 2018;50(8):859–882. doi: 10.1002/lsm.22823.
- Wang Y. Red (660 nm) or near-infrared (810 nm) photobiomodulation stimulates, while blue (415 nm), green (540 nm) light inhibits proliferation in human adipose-derived stem cells. Sci. Rep. 2017;7(1):1–10. doi: 10.1038/s41598-017-07525-w.
- Wang Y. Photobiomodulation (blue and green light) encourages osteoblastic-differentiation of human adipose-derived stem cells: role of intracellular calcium and light-gated ion channels. Sci. Rep. 2016;6:33719. doi: 10.1038/srep33719.
- Ermakov A.M. Opposite effects of low intensity light of different wavelengths on the planarian regeneration rate. J. Photochem. Photobiol. B Biol. 2020;202:111714. doi: 10.1016/j.jphotobiol.2019.111714.
- Martel A., Liberman J. Inner Traditions/Bear; 2018. Light Therapies: A Complete Guide to the Healing Power of Light.
- Gottlieb R.L., Wallace L.B. Syntonic phototherapy. Photomed. Laser Surg. 2010;28(4):449–452. doi: 10.1089/pho.2010.9933.
- Spitler H.R. College of Syntonic Optometry. Reprint; Eaton, Ohio: 1941. The Syntonic Principle; p. 1990.
- Hanselmann R.G., Welter C. Origin of cancer: an information, energy, and matter disease. Front. Cell Dev. Biol. 2016;4:121.
- Prinsloo, G.M. 2011. A device for coherent and/or non- coherent irradiation of human bodies with therapeutic effects. Patent number WO2012035363.
- Antonovsky A. vol. 175. 1987. Unraveling the Mystery of Health. San Francisco.
- Rubin R. Wolters Kluwer Health/Lippincott Williams & Wilkins; 2011. Rubin’s Pathology: Clinicopathologic Foundations of Medicine.
- Porges S.W. The polyvagal theory: phylogenetic substrates of a social nervous system. Int. J. Psychophysiol. 2001;42(2):123–146. doi: 10.1016/S0167-8760(01)00162-3.
- Suh S. The expression of opsins in the human skin and its implications for photobiomodulation: a systematic review. Photodermatol. Photoimmunol. Photomed. 2020 doi: 10.1111/phpp.12578.
- Vardhana S.A., Wolchok J.D. The many faces of the anti-COVID immune response. J. Exp. Med. 2020;217(6) doi: 10.1084/jem.20200678.